David J. Linden is not a fan of books about the brain written by non-scientists. The Johns Hopkins professor of neuroscience, himself the author of some popular books on the mind, says he finds too many dull, inaccurate, sometimes even fraudulent volumes on the shelves of bookstores, works that at best are boring to read and at worst contribute to what he terms "a firehose of neurobullshit." To remedy this situation, Linden reached out to his scientific colleagues, 13 of them at Hopkins, asking them to write essays on what they'd most like the public to understand about the human brain.
The result is Think Tank: Forty Neuroscientists Explore the Biological Roots of Human Experience, just published by Yale University Press. Four of the essays by Johns Hopkins researchers are excerpted here.
Children's brains are different
by Amy Bastian, professor of neuroscience at the Johns Hopkins School of Medicine
I recently went skiing for the first time in many years. I was out of shape and definitely out of practice, so I began by carefully working my way down one of the easier slopes. Suddenly, out of nowhere, a tiny child zipped right in front of me. I lurched out of the way and fell, losing a ski and a pole in the process. I was initially annoyed—where was this kid's parent? After my frustration subsided, I made some effort to watch tiny children rocket down steep hills. How on earth do young children learn to ski so well?
Very young children appear to learn many skills better than adults, in such areas as sports, languages, and musical instruments. In fact, most coaches and teachers will tell you that you must start young if you are going to become, for example, a great tennis player or a concert violinist. And you typically have to start young if you want to learn a second language as proficiently as a native speaker.
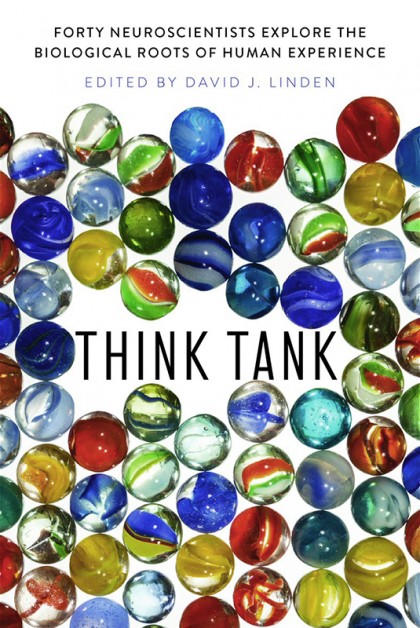
What is special about a child's brain? Ask almost any neuroscientist, and he or she will probably say, "A child's brain is more plastic." This answer is not terribly helpful because it does not address what "plastic" means, what makes a brain plastic, or why it becomes less plastic in adulthood. Here I will use a simple definition of "plasticity": the ability of the brain to change its own connections and functions as a result of new experiences.
One of the most dramatic aspects of brain development that could underlie plasticity occurs in infancy and early childhood and involves a massive proliferation of neural connections. The brain of a two-year-old child has twice as many neural connections as the adult brain. The number of contacts between neurons (i.e., synapses) explodes during infancy, with some estimates suggesting that hundreds of new synapses are formed every second! This abundance of neural connections is eventually pruned back throughout childhood and adolescence to reach adult levels.
One important influence on whether connections stay or go is whether those connections are used. Thus the variety, intensity, and type of experiences of infants and young children are incredibly important for their developing brains. Connections that are used as a child moves, listens, sees, thinks, and feels are the ones that are more likely to stick. Without doing these things, the connections may be weakened or removed.
It is also important to define what constitutes heightened learning ability in children compared to adults. Children are superlearners in the sense that they can learn to be more proficient compared to adults—that is, they can gain fluency in a second language that is comparable to that of a native speaker. But this does not mean that every aspect of language learning is better. In fact, children learn a second language more slowly than adults. Similarly, it appears that younger children learn new movements at a slower rate compared to adults. Some work in this area has shown that this motor learning rate gradually improves (i.e., speeds up) through childhood and becomes adult-like by about age 12.
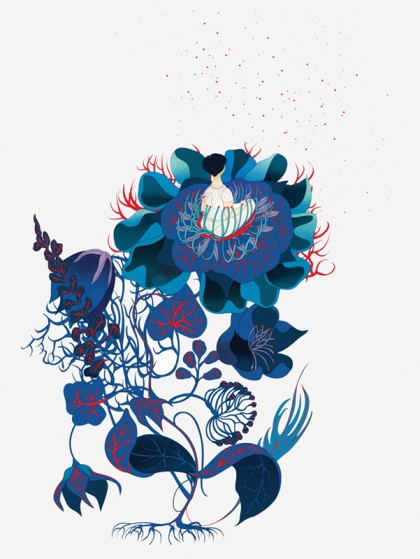
Image credit: Marcos Chin
If children learn more slowly and are more variable in their movements, why do they appear to learn certain tasks like skiing better than adults? First, children are smaller than adults; thus their center of mass is lower, a factor that may make activities like skiing easier to learn to control. However, this factor would not explain their learned proficiency across fine motor skills, such as playing a video game, an activity that involves only hand movements. Second, the variability in children's movements might also work to their advantage, as they get to try out many different ways of moving for a given situation in order to find the best one. Adults may be less willing to explore different movements and therefore tend to settle on a suboptimal motor pattern. Third and perhaps the most important factor is that children may be more willing than adults to undergo massive amounts of practice to learn motor skills. For example, when infants are learning to walk, they take about 2,400 steps and fall seventeen times for each hour of practice. This is an intense amount of activity; it means that infants cover the length of 7.7 American football fields per hour!
Unfortunately, there is also a downside to experience-dependent plasticity in childhood because any kinds of experiences affect brain development, not just the positive ones. So although plasticity can make children learn better, it can also cause problems. Stress and negative experiences can lead to maladaptive changes in a child's brain. For example, young children who experience events such as neglect, abuse, or poverty have an increased risk of developing problems such as anxiety, emotional dysfunction, and cognitive deficits.
Ultimately, all experiences, as well as the lack of them, count a lot during early development. Children can take advantage of early plasticity to learn many things better than adults, including zipping down a ski slope and speaking French. But this plasticity can also put children at risk when they have negative experiences or are deprived early in life. Scientists don't fully understand the processes that contribute to childhood brain plasticity, but it seems clear that early life experiences are incredibly important.
You have a superpower—it's called vision
by Charles E. Connor, professor of neuroscience at the School of Medicine
For those gifted with normal sight, vision is a superpower. At a glance, you can tell where you are, what is around you, what just happened, and what is about to happen. You effortlessly perceive the precise three-dimensional structure of objects in your environment at distances ranging from millimeters to miles. You know what they are called, how valuable they are, how old or new, fresh or rotten, strong or weak. You effectively read the minds of other humans and animals based on tiny variations in facial configuration and body pose.
Understanding what you see seems trivial—you only have to look at it! But imagine that you travel to a world of sentient, humanoid creatures without vision; call them Glorbons. These creatures possess our other sensory and motor abilities, as well as comparable intelligence. They understand and interact with their environment based on touch, hearing, taste, and smell. Think how magical your visual abilities would seem in such a world. Your immediate, accurate descriptions of all the things in their houses and cities, regardless of distance, things they know only through laborious close-up exploration, seem supernatural. You have an oracular ability to forecast physical events like oncoming storms. Your ability to recognize individuals from afar and intuit their health, mood, age, and intentions before they even speak resembles witchcraft.
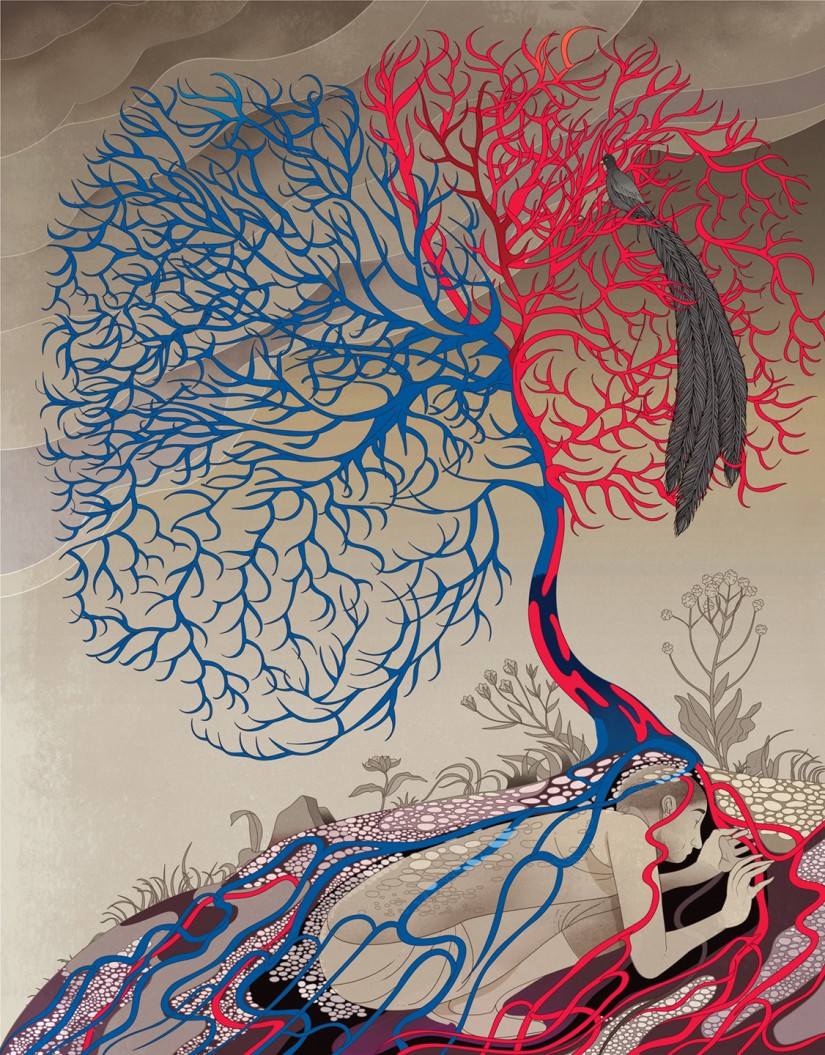
Image credit: Marcos Chin
You try to demystify this ability to the Glorbons by teaching them about how vision works. Drawing on your dimly remembered high school physics, you explain that vision is based on photons—particles, or maybe waves, that weigh absolutely nothing and travel through space at about a billion feet per second. You tell them how the paths of photons can be bent by the lens in your eye to form an image, a two-dimensional map in which each point on the retina, the layer of light-sensitive cells at the back of the eye, receives photons only from a corresponding direction in space. "Okay," respond the Glorbons. "What do you do then?" "Well," you reply, "then you just see what is in the image." But the Glorbons don't understand what "seeing" means. And as you try to explain, you begin to realize that you don't understand seeing either.
Promising the Glorbons that you will return, you travel back to earth, where first you consult an expert on computer vision, Professor Y. He shows you a project outline from MIT in the 1970s, predicting that computer algorithms for recognizing objects in photographs could be designed by summer students. But now, he says, nearly 50 years later, it remains a largely unsolved problem. Professor Y takes you to his lab to see AlexNet, a standard deep computer network, in action. He brings up a picture of a penguin standing in a snowy field, with some other penguins and mountains in the background. The network draws a box around the penguin and labels it with a 70 percent probability that it is a bird. Pretty good, you think, though you personally would have given it a 100 percent chance of being a penguin.
Next, Professor Y adds a computer monitor into the picture, right next to the penguin. You start to laugh at the Monty Pythonesque incongruity, but then you notice that the network has now labeled the penguin with a 70 percent probability of being a person. How can that be, you say—it's obviously a penguin. Professor Y explains that this is how the most successful object identification programs work, using statistical inference on cues from the entire image to make a best guess. But, you say, what about the beak, the down, the wings . . . and then you realize that AlexNet doesn't have all of this overly complete information about the physical reality of the penguin. Instead, it learns the simplest, most reliable cues for object identification.
Clearly, you need someone who understands human vision, so you consult a visual neuroscientist, Professor Z. You tell her about your interest in learning how human vision works.
First, she tells you about anatomy—how perhaps half of the cerebral cortex in primates (including humans) is involved in vision in some way. The primary visual cortex, at the back of the brain, which receives visual information detected and processed by the retina and then further transformed by an intermediary structure called the thalamus, is just the start. From the primary visual cortex, information is distributed through dozens of other specific brain regions devoted to objects, scenes, faces and bodies, and to movement and color. Action-related areas use vision to decide what to look at next, how to navigate through the world, how to reach out and grab objects.
No wonder visual experience is so rich and information-dense, with half the cerebral cortex working on it every waking minute. But, you say, that is all about where vision happens. What I really want to know is how it works. "It's complicated. We've had a few successes; we probably know the most about visual motion perception—how groups of neurons signal direction and speed." Oh, you say, for example, how we appreciate the ballet? "Not quite; more like how we decide whether a field of dots is moving left or right."
Dejected, you walk away, deciding that visual neuroscience is full of profound questions but disappointing answers. Vision is a superpower, and it remains mysterious, for now. The Glorbons will have to wait.
Mind reading emerged at least twice in the course of evolution
by Gül Dölen, assistant professor of neuroscience at the School of Medicine
When we think of mind reading, most people imagine the abilities of a magician, an evil genius, or an alien life form. In fact, we perform mind reading every day. For example, as you are reading this [article], maybe you are on the subway, and there are no open seats, so you are standing. The train pitches and sways, and from the corner of your eye you notice that a nearby passenger starts to gather up his belongings. So without drawing too much attention to yourself, you move in closer. When the passenger gets up, you quickly swoop in and grab the seat.
In carrying out this simple strategic move, you have performed several acts of mind reading. Neuroscientists call mind reading of this sort "Theory of Mind," abbreviated ToM. ToM is a cognitive ability that is only a theory insofar as it is a best guess about another person's mental states (that is, desires, beliefs, knowledge) and an understanding that these mental states might be different from your own. As in the example above, we use this theory to understand other people's motivations, anticipate their actions, and modify our own behaviors accordingly.
Video credit: Dr. William Ashton
Consider, for example, the Heider-Simmel illusion. An animated film shows a large rectangle, a big triangle, a small triangle, and a small circle moving around a page. When asked to describe what the film is about, most people will ascribe thoughts, feelings, and emotions to the two triangles and the circle and construct a scenario explaining interactions among them—for example, the small triangle is annoying, the big triangle is a bully, the circle is a dreamer.
Many scientists have held that ToM abilities are restricted to humans. Anyone with pets, especially dogs, would be incredulous at this assertion because so much of the behavior exhibited by these companion animals seems to meet the criteria for having a ToM. There are three theoretical problems for scientists wanting to formally claim that animals have this attribute.
First, reflexive mind attribution is difficult to turn off, even when we know the objects (such as circles, triangles, dolls, or figurines) are incapable of desires, beliefs, or knowledge. Thus, when objects are not objects at all but living, breathing companions, it is hard to know whether we see feelings, emotions, and thoughts in our pets because they really have them or because our brains are wired to believe that they have them.
The second problem for scientists who want to argue that animals have a ToM is how to demonstrate it to a skeptic. For example, we might be tempted to say that a dog brings you his leash when he wants to be taken out because he anticipates that your inability to find it will delay departure. A skeptic might argue that this behavior is just a sequence of actions that the dog has learned to produce the desired outcome.
The third problem for animal ToM is that in terms of brain anatomy, only humans are thought to be equipped with the required brain regions implicated in ToM.
It is unlikely that ToM abilities in humans emerged spontaneously. Instead, they probably evolved from existing cognitive abilities. We humans mainly use ToM in a social context; therefore most researchers believe that this cognitive function evolved in response to the selection pressures imposed by social living. By this reasoning, ToM-like abilities might only be observed in species that live socially (for example, humans, most primates, dolphins, and wolves).
Video credit: SciNews
Nevertheless, we can imagine that the ability of a predator to catch its prey would be greatly enhanced if the predator were to attribute mindfulness to the prey. This idea raises the interesting possibility that predatory rather than social selection pressures could have shaped the evolution of ToM. If so, ToM-like abilities would be much older (in terms of evolutionary time) than has previously been supposed since hunting almost certainly evolved earlier than social living. Supporting this latter view, the recently rediscovered larger Pacific striped octopus exhibits a unique hunting behavior that suggests that these animals might have ToM-like cognitive abilities. When hunting shrimp, this octopus uses what appears to be a variant of the shoulder-tap prank. Having identified its prey, the octopus assumes a dark-colored skin pattern and then very slowly extends one of its dorsal arms, arching it above and around the shrimp. When the octopus is in position, it lowers the tip of its extended dorsal arm behind the shrimp and taps it on the posterior of the shrimp's body, causing the shrimp to leap forward into the octopus's other seven arms.
Still the problem of brain anatomy remains: The octopus brain is nothing like a human brain. It doesn't have any of the cortical regions suggested to underlie ToM or even, for that matter, a cerebral cortex. Nevertheless, the degree of encephalization and neuronal expansion seen in octopuses sets these species apart from other invertebrates. Thus although octopuses don't have cortical regions associated with ToM, they have an exceptionally large brain capacity and may have evolved to solve the problem of ToM using different anatomical strategies.
Perhaps the most effective way to determine whether octopuses have ToM would be to show that the same genes that are necessary for this attribute in humans are also necessary for ToM-like behaviors in octopuses. Experiments to test this possibility are underway in my lab. Recently, a set of genes has been described that controls language in both humans and African grey parrots, despite anatomical differences in brain organization between humans and birds and the absence of a common ancestor that shares the language trait.
Almost everything you do is a habit
by Adrian M. Haith, assistant professor of neurology
Do you check your smartphone every few minutes? Maybe you bite your nails or eat too much chocolate. Or perhaps you go to the gym regularly and always wash up straight after dinner.
Habits, good or bad, are a fact of life. We all perform some actions habitually without actually thinking about them. Sometimes these actions are undesirable, and we resent their intrusion into our otherwise thoughtful and deliberate existence. Others exert a positive influence on our lives, and we deliberately cultivate them as a force for self-improvement. Although it may seem that just a few aspects of our daily behavior might be described as "habitual," the truth is that beneath the surface of our cognition lurks a vast and unseen conglomeration of habits that drives almost everything we do.
For the purposes of scientific inquiry, the definition of "habit" as a thoughtlessly generated action is rather too loose. Neuroscientists have therefore adopted a definition of "habit" that is more precise and measurable. The definition depends on the idea that a person or animal always has a goal. For example, our goal might be to drive to the grocery store to buy milk. In pursuit of this goal, every decision we make or action we choose should ideally be one that brings us closer to this goal. If so, our behavior is said to be goal-directed.
By contrast, if our choice of action is the same regardless of our goal, then our behavior is habitual. If your goal is to pick up milk on the way home from work, you might nevertheless habitually drive straight home. What distinguishes habitual from goal-directed behavior is not the suitability of your action choices but whether or not they adapt when your goals change.
Once you begin thinking of habits in terms of inflexibility rather than utility, it does not take long to identify examples that illustrate just how habitual our everyday lives are. Consider the simple act of sending a text message on your phone. Its true habitual nature will be betrayed by a change in circumstances, such as when you buy a new smartphone. First, you must unlock the phone—a simple enough task until new software or hardware requires you to push a different button or execute a different kind of swipe, in which case your habitual actions frustratingly lead you to instead change the volume or enter camera mode.
Habitual and goal-directed behaviors have been shown to have distinct underlying neural bases. Through the kinds of navigation experiments in rats described above, it has been found that habitual behavior and goal-directed behavior depend on distinct underlying brain circuits. Both types of behavior depend on an interaction between the cortex and the basal ganglia but on different subregions of these structures. The dorsomedial striatum, a subregion of the basal ganglia, seems to be critical to goal-directed behavior. If it is damaged, the rat's behavior will quickly become habitual even after its limited exposure to a new task. Conversely, damage to the neighboring dorsolateral striatum will lead the rat to be more goal-directed and it will never lapse into habit, even after prolonged training. This finding suggests an intriguing, if perhaps drastic, solution to eliminating your unwanted habits. If you could inactivate just the right part of your dorsolateral striatum, you could be rid of them for good. Who wouldn't want to be goal-directed all the time?
Although it is true that the inflexibility of habits is a major drawback, this is outweighed by certain decisive advantages. Primary among these is their thoughtlessness. This advantage can be very important as behavior gets more complex. A hunter who no longer has to deliberate about how to launch his spear will be better able to track and anticipate the movements of his prey. Furthermore, the habit of throwing a spear can be deployed rapidly and automatically, without the need to wait for time-consuming thought processes. Since most circumstances in the real world are predictable and repetitive, the inflexibility of being habitual rarely matters.
There appears to be no limit to the number and complexities of habits we are capable of acquiring. Professional tennis players and soccer players must combine precise technique with awareness of other players' movements and make rapid, complex decisions about exactly what to do next. Such mastery can be achieved only if the basic elements of their skills (like correct technique), and even many of the more complex elements (like choosing the right pass or shot at the right time), have been forged into habits through years of practice.
Impressive as elite athletes may be, we should not underestimate that our own abilities to walk, talk, read, write, type, tie our shoelaces, do mental arithmetic, operate a phone, and play video games are extraordinary accomplishments in their own right. We all share a remarkable repertoire of learned behaviors, acquired through a lifetime of practice. As each new ability is learned and consolidated into habit, we move on to mastering the next one, building a vast library of skills that can be invoked in an instant, enabling our thoughts and actions, in all their complexity, to operate mostly on autopilot. Atop this mass conglomeration of habits sits a thin sliver of cognitive deliberation that steers only the highest-level decisions that we ever need to make. Without habits, it would be quickly overwhelmed.
Posted in Health, Science+Technology
Tagged brain science, neuroscience, cognitive science, neurology