Molecular biologist Geraldine Seydoux tinkers with worm DNA like a home stager preps a living room. Students call her the worm guru, but to a primitive organism called C. elegans, a transparent nematode that grows to about 1 mm in length, she's more or less a god. Using CRISPR-Cas9, a revolutionary gene-editing technique, Seydoux can delete, replace, or reprogram a nematode gene at will—and then step back and observe the result.
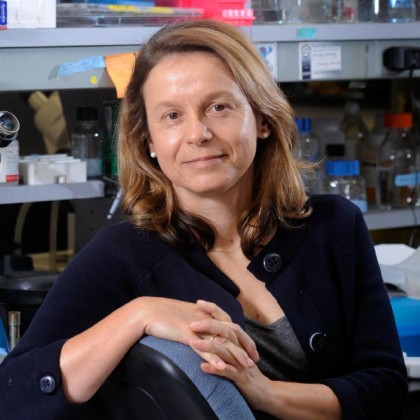
Image caption: Geraldine Seydoux
We've known since the discoveries of Augustinian monk Gregor Mendel in the 1860s that genes are the basic drivers of heredity, an organism's assembly instructions. Each gene codes for RNA, and RNA in turn determines the folded structures of proteins, the biomolecules that function as the worker bees of an organism's cells. Interfere with a gene, and a protein no longer will do its job, or will do it differently.
Applying CRISPR, Seydoux can edit genes to make a worm stop reproducing. She's made them glow in the dark under special lights by combining their genes with jellyfish DNA. This was not a parlor trick—she wanted to see how certain proteins dispersed in a cell. Her lab recently knocked out a gene that left the worms unable to produce a specific spot for storing RNA. To the worm, that was a big deal because it degraded its ability to fight off foreign DNA. "I go in and change their code and see what happens," says Seydoux, vice dean for basic research at the School of Medicine. Speaking of adenine and cytosine, two of the four nucleotides that are the basis of the genetic code, she says, "Let's change this base A to nothing, or replace it with a C. This helps me understand what this protein does. If I change the code and now the worm is missing a tail, well, now I know this protein is important to make a tail. Using a tool like CRISPR to make any mutation we want, big or small, speeds up our ability to decode the genome, to figure out the function of every gene. That's a major goal of biology."
Seydoux says it's far from hyperbole to say CRISPR has revolutionized genetics. What used to take months or even years to create and observe can now happen in weeks or days thanks to CRISPR, Science magazine's 2015 Breakthrough of the Year. Whose breakthrough? That's a hotly debated topic. There's currently a patent war raging between MIT neuroscientist Feng Zhang and the pair of Jennifer Doudna, a University of California, Berkeley, biologist, and Emmanuelle Charpentier, a French microbiologist now with the Max Planck Institute for Infection Biology in Berlin. MIT filed for a patent in 2014, but Doudna and Charpentier proved that CRISPR tech was viable back in 2012. A Nobel Prize awaits someone. CRISPR is faster, cheaper, and more accurate than previous techniques for editing DNA and has potential applications well beyond just messing around with nematodes. Scientists like Seydoux can edit genes in any animal. Including humans.
Researchers across the world have already used the tool to engineer viruses that kill antibiotic-resistant bacteria, modify immune cells to destroy cancer, genetically alter pigs, make mushrooms that resist browning, and muck around with the color of butterfly wings by editing its "paintbrush" gene. In mouse studies, scientists have used the tool to correct the genetic errors responsible for sickle cell anemia, muscular dystrophy, and cystic fibrosis. One group was successful in snipping HIV DNA from the genome of live mice, and now wants to attempt the process in primates. At Johns Hopkins, a team at the Wilmer Eye Institute is using CRISPR to edit retinal ganglion cells that could be transplanted into a patient suffering blindness caused by glaucoma or multiple sclerosis. A group in the Bloomberg School of Public Health is looking to modify mosquitoes so that the bug's offspring won't transmit malaria to humans.
In talking with scientists familiar with the system, one overriding sentiment emerges—this is one very cool technology. "CRISPR is an amazing tool for research," Seydoux says. "And it's an amazing aspirational tool for clinicians because they can potentially go in and fix mutations in humans." It just might live up to the hype, leading us to a day when we can cure genetic diseases. But there are concerns. Should anyone be allowed to play god and tinker with the human genome to alter genes in embryos, sperm, or eggs in ways that can be passed from generation to generation? Many critics fear the prospect of designer babies, a future like that in the movie Gattaca, in which man can modify embryos to produce people with enhanced musculature, intelligence, beauty, and other desired traits. That day may become reality, but first there are many technical and ethical hurdles to jump. Seydoux and other basic scientists are part of a camp that says let's not skip ahead to fear of a dystopian future. CRISPR has settled into a space where it's a useful, ubiquitous tool that is speeding up science and our understanding of the human genome and genetic diseases. Let the fine-tuning of this still-fledgling technology continue.
In her office at the Pre-Clinical Teaching Building, Seydoux erases an exquisite and byzantine formula of numbers, letters, squiggles, and lines from a dry-erase board. She wants to demonstrate how CRISPR works. First off, she says, CRISPR stands for "clustered regularly interspaced short palindromic repeats," which certainly justifies the acronym. She draws some random DNA code: AAGGTTC. The DNA that makes up our genome is composed of such sequences of adenine, thymine, guanine, and cytosine: A, T, G, and C.
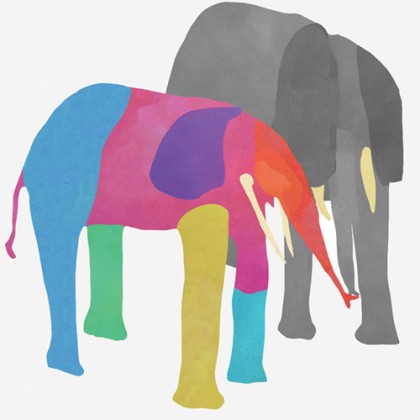
Many liken CRISPR to a word processor's search-and-replace function, applied to editing those vital sequences. The first step: Take a protein called Cas9, derived from bacteria that cause strep throat. The natural function of Cas9 is to kill invading viruses, which it does by snipping the invaders' DNA. So think of Cas9 as molecular scissors. Now you need a way to guide those scissors to a precise place in a cell. That's where guide RNA, known as gRNA, comes into play. Guide RNA that has been human-engineered for this purpose acts like a DNA-sniffing dog, helping Cas9 find an exact DNA sequence and snip it out. To demonstrate, Seydoux erases a few letters from the AAGGTTC on the board. "You see? Boom. Gone."
Seydoux makes her own Cas9, a clear, purified extract from its host bacteria that is then stored in tubes and refrigerated. Once she programs the Cas9 with the gRNA, she can inject it into whatever cell she wants. Sometimes CRISPR makes a mistake and finds a gene that is similar but not identical to the target, and cuts there. "But that is becoming very rare," she says.
Seydoux says CRISPR is faster than any previous technique because researchers can work directly in animals. Before CRISPR, to make a mouse with a desired mutation, one would first have to draw out certain cells from a mouse embryo, engineer those cells with the mutation, then put them back into the embryo, a process that took at least a year. "Now, we can create the mutation directly in embryos, in just 21 days," she says. The mouse passes down this mutation to its offspring, and in a few months an entire line of lab mice or worms or other model will all carry the same mutation.
Within a year of its discovery in 2012, CRISPR was being used in nearly every molecular genetics lab across the nation and the world, says Nathaniel Comfort, a professor in the Institute of the History of Medicine at the School of Medicine. Comfort, author of The Science of Human Perfection: How Genes Became the Heart of American Medicine, wrote in a July 2015 essay in The Nation that once the kinks are worked out, "CRISPR seems likely to change the way biologists do experiments, much as the circular saw changed how carpenters built houses." Two years later, he says, this vision has mostly come to pass.
"I think the story about CRISPR is less the sexy and scary future scenarios, and more about how it has changed how scientists work," says Comfort, whose next book will be a biography of DNA. "It's literally changed the face of science. It's all about speed and cost. It democratizes genetic engineering. Now any grad student can tweak a gene, go get some coffee, and, bam, it's done."
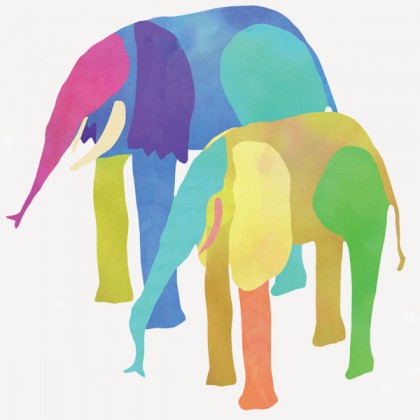
David Valle, director of the McKusick-Nathans Institute of Genetic Medicine and professor of pediatrics and ophthalmology at the School of Medicine, says that many labs at Johns Hopkins adopted the new technology early on; now it's become standard practice. Anyone with a basic level of science, he says, can use it. One startup company, called The Odin, sells CRISPR kits that any would-be biohacker can use to tinker with yeast or bacteria genes in their basement. The Odin's CEO and founder, Josiah Zayner, a former research fellow at NASA, claims he's already edited his own DNA and cured himself of irritable bowel syndrome.
Joel Pomerantz, an associate professor of biological chemistry in the School of Medicine, was one of the early adopters of CRISPR at Johns Hopkins. In 2013, he used the technique to create a desired amino acid substitution in a protein that was discovered to play a role in the immune system. He injected CRISPR-modified components into 118 single-cell mouse embryos, which were then implanted in female mice. Of the 23 pups born, four contained the desired change. Back then, that was considered an extremely high rate of success, he says, and the best part was it took only four months to go from project conception to his desired mutant mice. This early success demonstrated to Pomerantz and his colleagues CRISPR's ability to rapidly generate mutations in order to learn about a protein's roles in immunity and cancer. "I'm not using CRISPR to try to cure anything," he says. "I'm just using it as a tool. But it's an incredibly powerful tool for almost anything in the biomedical science realm."
Before CRISPR, Pomerantz says, it would take on average two years, and in the realm of $30,000 to $50,000, to produce the desired mouse strain. "With CRISPR, we can now create this mouse strain in four months for, like, $6,000. That's one aspect that has really impacted the work in our lab. You don't think anymore, 'Oh, do I have time for this experiment, or can I get the money for this?' You can just do it. It's amazing."
Another prized aspect of CRISPR is it gives researchers the ability to screen for unknown roles of genes, whether it's the ability to fight off a pathogen, influence growth of lymphocytes, or make healthy tissue turn cancerous. With CRISPR, scientists can make libraries of gRNA for every gene, and researchers can develop a system to assess the effect of single genetic changes. For example, Pomerantz is studying a signaling pathway that controls a gene regulator involved in the development of lymphoma. In one experiment, he and his colleagues monitored the effects of a mutation that led to abnormal growth of blood cells that stopped short of full-blown cancer. "What that tells us is that you need additional mutations to give you this specific cancer we're looking at," he says. "So now the question is, what other ones? We will use this library of guide RNA to set up a system so that we can mutate every other gene and see which ones cooperate with our existing mutation to give you the cancer." Once they discover these mutated genes, whether it's two, three, or four, researchers can develop drugs or other therapies to target those genes.
Andrew Holland, an assistant professor of molecular biology and genetics at the School of Medicine, dedicates much of his work to the study of cell division. His lab is particularly interested in how cells apportion genetic information during division to ensure that each daughter cell contains all the instructions required for further growth and development. What are the consequences to the cell and organism if errors were to happen during division? For Holland's lab, genomewide CRISPR screens have been instrumental in researching how the cell recognizes any irregularities during division and signals for cell cycle arrest.
"The [CRISPR-related] stuff going on here at Johns Hopkins is not stuff that would make you afraid," Pomerantz says. "It's the natural acceleration of the excellent work everybody is already doing to understand better his or her questions. How does diabetes happen? How does cancer happen?"
Much of the excitement centers around CRISPR's potential to treat or eradicate genetic disorders. Just one little change to a gene can manifest a disease like cystic fibrosis, Down syndrome, sickle cell disease, or hemophilia. Maybe correcting that change could offer a cure.
Scientists expect CRISPR to succeed first against a relatively rare, single-mutation disease such as hemophilia, which impairs the blood's ability to clot. In 2016, researchers from the University of Pennsylvania developed a gene therapy approach to treat hemophilia B in mice. The treatment involves inserting a vector that will deliver Cas9 to the liver in order to fix the clotting error.
Valle says that developing potential therapies means first figuring out how a particular mutation causes something like hemophilia. He studies Mendelian diseases, in which inherited mutations in single genes can cause sickle cell anemia, Tay-Sachs disease, or cystic fibrosis. "If I understand how the genetic variant produces the disease, then I can envision some rational treatment," Valle says. "This gene encodes for this protein that works in this biological system, and this disease or disorder occurs when this biological system is not functioning properly."
Seydoux says the pioneering work of Valle, and of the Johns Hopkins medical geneticists Victor McKusick and Barton Childs before him, will dovetail with the work of basic scientists like her using CRISPR. "When someone like a Dave Valle tells me, 'I have this weird mutation in this gene, I don't know what it does, but it causes this disease in humans,' I can say that I've studied this gene in my worms for 20 years, and I know it's important to do X. We're decoding the genome; he's figuring out where problems arise. We put that information together."
Treating genetic disease, Valle says, will still be a complicated endeavor. Making a change to a gene in cells in a petri dish is a lot different from introducing that change to a human. Consider a protein with 500 amino acids. The gene that encodes that protein has a mutation that alters the amino acid at position 393, which results in a malfunction of the protein. To confirm that this mutation actually causes the disease, studies in an animal model are often required. If that research confirms that this mutation is responsible for the disease, can we use gene editing techniques to correct the gene in human patients? In what tissues should this correction be performed, and at what developmental time? What level of efficiency is needed to restore normal physiology? Ten percent? Ninety-nine percent? "These are all technical challenges that will require additional research, may be difficult to overcome, and likely will vary for each disease," Valle says.
In editing the human genome, scientists must worry about unintended consequences, changes to other parts of the genome that are called off-target effects. At present, there's no infallible way to cut out—or edit—one mutation in a person's DNA using CRISPR without making a number of unintended edits elsewhere. Trying to eliminate a harmful mutation could lead to cancer or hampering a previously normal biological system. Fix one thing, we've broken something else.
Comfort says part of the problem is the still cavernous hole in our knowledge of the human genome. "We shouldn't pretend we know all the 'genes for cancer,' or all the 'genes for intelligence.' What we do have is some knowledge of which genes produce which proteins and parts of proteins, which do a lot of different things in different parts of the body," he says. "We have some serious kinks to work out. We still have some pretty big issues with accuracy and penetrance. Some genes we manipulate can only have very small penetrance—they might affect 3 to 5 percent of the variance for a given trait. And we're still working on what we mean by 'traits.' That's been a problem for geneticists for the past 150 years."
In the spring of 2016, a group of 150 scientists held a closed-door meeting at Harvard Medical School to discuss a project to create a human genome from scratch in a lab. That same year, a Chinese group became the first to inject a person with cells that contain genes edited using the CRISPR-Cas9 technique. The researchers altered immune cells from the recipient's blood, hoping that the edited cells would attack and defeat a cancer. The team has not yet released results of the trial.
This past summer, a team of researchers at OHSU in Portland, Oregon, used CRISPR to alter a human embryo, with limited off-target mutations. They were able to insert CRISPR into eggs at the same time they were fertilized by sperm. This technique lessened the risk of what's called mosaicism, when the desired DNA changes are not taken up by all the cells of an embryo, only some. None of the embryos were used to create a baby, and they were immediately disposed of. Still, this kind of research worries some people. Any changes made in the DNA of an embryo could be passed down for generations, which raises fears that human-induced mistakes could become a permanent part of humans' genetic inheritance.
"That is why people are more comfortable with using CRISPR in human tissue, not the germline," says Seydoux, referring to the genetic material in a cell lineage. "Right now, to my knowledge, there is really no reason to make CRISPR mutations in humans that will be passed on to the next generation. I see zero reason for that." Skeptics have warned of a new, CRISPR-based eugenics that could be as evil as any historical form of eugenics.
Comfort says it's pointless to wring our hands and say scientists shouldn't edit the human genome because it's happening already. While the Louise Brown of CRISPR doesn't exist yet, referring to the English woman known as the first human born through in vitro fertilization, Comfort says germline-edited people will be walking around at some point in the future. "How do we minimize the impact?" he says. "I'm about harm reduction. I'm not a bioethicist trying to tell people what they ought or ought not do. But I want to make sure this technology harms the smallest number of people. Let's try to make sure we actually understand this gene's total role in the body before we go half-cocked and edit or knock it out."
There are other proven ways to keep people healthy, Comfort says. Improve nutrition. Improve schools. Reduce violence. Equalize opportunity. He says we can't forget those. "We can pursue these cool technological options, too. And I agree, they're cool. I'm a huge science fan—but I'm also a science skeptic," he says. "I'm wary of how we sometimes say it's science, so it must be right. But let's evaluate and look at it critically. If we modify this gene because one study says it raises your IQ 7 points, does it also increase your chance of disease? Those are hard things to know. Biology is hard. That's one of the reasons I worry about the hype. Even if you have no hope of stopping the car, you can at least put the brakes on so it doesn't go careening off the embankment."
In November 2016, Pomerantz and Debra Mathews, assistant director for science programs at the Berman Institute of Bioethics, hosted a Baltimore Science Cafe at a Baltimore bookstore to discuss CRISPR and the impact of genome editing. The event drew a standing-room-only crowd of nearly 100 people, a mix of Johns Hopkins students and science-interested Baltimore residents. "Ninety-nine percent of what we talked about were the ethical implications," Pomerantz says. They asked questions about the potential to modify the human genome, make designer babies, and correct inherited diseases. Many in the audience wondered, Pomerantz says, how the technology could be monitored and regulated, and who should regulate it. The FDA? Some other governmental agency or council? What if other countries don't regulate it with all the same safeguards as us, and allow the human genome to be irrevocably altered?
Mathews, who is also an associate professor in the Department of Pediatrics at the School of Medicine, published a paper in Nature in November 2015 called "CRISPR: A path through the thicket." In the paper, Mathews and colleagues laid out some key points for consideration as various advisory bodies, scientific organizations, and funding agencies deliberate genome editing in humans. The paper came on the heels of a meeting of the Hinxton Group—a network of scientists, ethicists, policymakers, journal editors, and funders—to address the ethical and policy issues around the editing of the human genome in the early stages of development and in germline cells. Should genome editing be allowed in basic research involving human sperm, eggs, and embryos? What safety precautions should be met before we consider it? And what uses for genome editing in human reproductive applications might be permissible? "We wanted to create a set of defensible recommendations and establish a model regulatory framework that could be adopted internationally. Develop a road map for basic research," Mathews says.
Mitochondrial replacement therapy has emerged as a promising tool to treat individuals with disease caused by mutant mitochondrial DNA. But the method is controversial because of its use of nuclear DNA from a patient, inserted into a donor's egg, thus creating an infant with, in a biological sense, three parents. One alternative to this therapy is the selective elimination of mitochondrial mutations by CRISPR gene editing, basically leaving behind the healthy mitochondria. But this process involves the risk of off-target mutations, creating new problems in the germline.
Animals are already in the crosshairs of gene editing. A Harvard team wants to resurrect the woolly mammoth by using CRISPR to edit the embryo of endangered Indian elephants to have "mammoth" traits. One U.S. company wants to genetically modify pigs so their organs have no trace of PERVs—remnants of ancient viral infections found in the pig genome that could infect a person who one day receives a pig's heart, lung, or kidney as a temporary or replacement organ. "Harvesting organs from pigs to transplant into humans is theoretically possible. But then there's the ethical question of the use of the animal in this circumstance, and a question whether this is truly feasible," Mathews says. "I'm not necessarily interested in shutting things down. I'm interested in designing research and medical interventions in ways that maximize benefit and minimize harm. I am concerned with who decides what goes forward, and how. We don't understand enough about genetics to know exactly how a genome or an organism will be affected when I modify my gene of interest."
Seydoux says that while her work sometimes might not be good for the worms, it's informative for science. "I think it is very important to remember how CRISPR was discovered—curiosity-driven science," she says. "Not science aimed at any particular practical goal. The biggest discoveries in biology all have roots in curiosity-driven science. I'm just trying to figure out how stuff works. Whatever question we feel like asking. That is what we will continue to do," she says.
Snip goes more worm DNA.
Posted in Health, Science+Technology, Politics+Society
Tagged genetics, berman institute of bioethics, crispr