In 1982 William DeVries, a cardiac surgeon at the University of Utah Hospital, successfully implanted an artificial heart in a patient who was suffering from end-stage heart failure. The recipient lived for 112 days with the device, designed by Robert Jarvik.
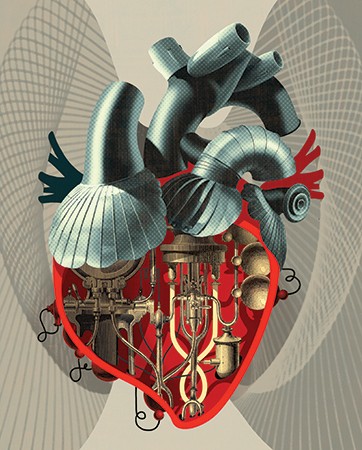
Image credit: Pierluigi Longo
Thirty years later, we've cloned sheep, developed the Internet, mapped the human genome, and progressed from LPs to CDs to MP3s, but we still haven't created an artificial heart that can sustain life for longer than a few months.
"If you think about technologies in general and how they've advanced in the past three decades, I don't think you'd say that artificial heart technology has progressed at a pace that's appropriate for the amount of time that has passed," says T.E. "Ed" Schlesinger, dean of Johns Hopkins' Whiting School of Engineering.
So what's the holdup? The challenges of creating an artificial heart that can "beat" an average of 35 million times a year for multiple years like a real heart are myriad. There are problems to solve regarding biocompatibility, power supply, blood flow, pumping systems, control mechanisms. Should the heart be fabricated from synthetic materials, muscle tissue grown from stem cells, or a combination of both? Does it have to pump like a real heart, or should it rely on a system of continuous flow, as current heart-assist devices do?
Last winter, more than 160 people from the Johns Hopkins community and beyond attended the first Hopkins Heart Symposium. The purpose was to kick off a 10-year, $100 million-plus collaboration between doctors, engineers, and systems experts at Johns Hopkins to build the world's first permanent totally artificial heart. It was a goal first proposed a year earlier by Duke Cameron, a professor of surgery and chief of Cardiac Surgery at the School of Medicine. William DeVries himself was the keynote speaker, while Johns Hopkins physicians presented talks on subjects with titles like "Heart Failure 101" and "Stem Cells and Tissue Engineering." Engineers spoke about the mechanics of pumping blood.
Since then, a team of medical researchers and engineers from across the university community has met monthly to brainstorm ideas and begin collaborating on research that will hopefully succeed where other efforts have failed.
"There is no better institution in the world than Johns Hopkins to see this initiative through," says Cameron, who serves on the project's executive committee. "Hopkins has broad expertise spanning clinical cardiology and surgery, biomedical engineering, and research in biological and physical sciences, plus a spirit of cooperation among disciplines that is unique among universities."
Also see: Getting pumped (JHU Engineering magazine, Winter 2015)
Currently, only one totally artificial heart is approved by the federal government for use in patients in the United States, but it has proved to be effective for only up to 18 months. In August, a French company, Carmat, implanted its second artificial heart, made from polyurethane and natural materials derived from bovine heart tissues, in a patient. (Its first recipient died 75 days after surgery.)
Tens of thousands of people are diagnosed annually with conditions that would benefit from new hearts, but because of a shortage of viable organs, only 2,000 to 2,500 transplants take place each year. More than 4,100 patients are currently on the national heart transplant waiting list. Like former Vice President Dick Cheney, many of them use a mechanical left ventricle assist device, or LVAD, as a bridge until a transplant organ can be found. Unfortunately, many die waiting.
"There is just not a sufficient number of organs to transplant everybody with significant heart disease who is eligible," says Gordon Tomaselli, a professor of medicine and chief of Cardiology at the School of Medicine, who has been involved in the initiative from its start. "This is the medical problem that we face, so we engaged the folks in Engineering and at APL to think about how we can, in a very multidisciplinary fashion, attack the various components of this problem. It's not just a single problem; it's a collection of problems, and many of them are engineering-related."
"It's a very difficult challenge," agrees Schlesinger. "It's a materials problem, fluid, mechanical, energy, medical. It's got so many different components. The question is, Which organization can bring together the array of expertise to address such a problem? I think, therein, Hopkins has a unique position."
Joe Katz is playing with a fabricated aorta in his second-floor office in Homewood's Latrobe Hall. Unfortunately, it's broken, sheared off at the left subclavian artery. "I have a lot of nervous energy, so I ended up breaking it, to the delight of everyone else in the room," he says sarcastically.
This is not a normal aorta, however. There's an exaggerated bulge off its left side—a major aneurism. "If this person doesn't get it treated and operated on, he's not going to live very long," he quips.
Katz admits he's a newcomer to the mysteries of the cardiovascular system. He's a mechanical engineer, a specialist in fluid mechanics who has made a name for himself by employing high-tech instruments to take measurements in a variety of fluid systems, from the ocean to the laboratory, with unprecedented accuracy. He's accustomed to testing turbines, propellers, jet engines—not blood flow. "I'm a pump guy," says Katz matter-of-factly. But a permanent artificial heart is the pump problem to end all pump problems. When then Dean of Engineering Nick Jones asked Katz to spearhead the engineering side of the Hopkins Heart Initiative, he signed on immediately.
In the last 18 months, Katz has set out on a journey to turn himself into a cardiovascular expert of sorts. He's picked the brains of colleagues in Engineering, met with Hopkins cardiologists, and sat in on open-heart surgeries. He's also spoken with patients hooked up to ventricle assist devices, asking them about their experiences. He found that while LVAD technology has improved in recent years, the devices still have their share of problems: Power packs can be bulky and uncomfortable for patients to carry about, the site where the power line enters the body is prone to infection, and up to 60 percent of the patients who receive them have to be re-operated on to control post-implantation bleeding.
But one of the biggest problems with LVADs, as well as with existing artificial hearts, is that they can damage the blood. Through shear stress, delicate platelets—whose function is to stop bleeding in normal situations—can become "activated," causing thrombosis or clots, which can lead to stroke or heart attack. It's the reason why patients require comprehensive anti-coagulation medication, which can have problematic side effects as well. Red blood cells can also be damaged by the high shear stresses caused by pumps and leach hemoglobin, causing more problems. So for engineers, physicians, and others working on the project, the mantra has become "Do not damage the blood."
"It's fundamental to the whole point," says Marty Devaney, a senior administrative manager in the Whiting School's Department of Mechanical Engineering, who's coordinating research efforts between Engineering, Medicine, and APL. "If we create an item that damages the blood, we're no better than any system out there right now, and we want to make sure we take into account the actual mechanism that does damage to the blood and limit that in our designs."
And while researchers have known that artificial pumps can corrupt the blood, they haven't pinpointed where in the devices the harm occurs. There was no existing data. So Katz and postdoc Jacopo Biasetti designed a "flow loop" to see if they could record the damage being done to platelets. Working with Thomas Kickler, a professor of pathology and director of Hematology and Coagulation Laboratories at the School of Medicine, they were able to "light up" activated platelets with a fluorescent material and record the results on video.
"We got some very interesting results," says Biasetti, who is, for now, the lone full-time employee working on the heart initiative. "Our aim in the next couple of months is to have an entire LVAD in our flow loop and visualize platelet activation and protein cleavage in real time on a real pump."
The university has signed nondisclosure agreements with two private manufacturers, ReliantHeart and Berlin Heart, to test their LVADs in experiments, with funding coming from NIH grants. The goal is to physically witness and record where the damage occurs—basic research that will help the Hopkins team in its own designs, says Biasetti, who's coordinating efforts between three labs—those of Katz, Kickler, and radiologist Assaf Gilad, where researchers will image in a similar system a blood protein called Von Willebrand factor, vital to platelet function, that can also be damaged.
"The idea is to come up with a format that should have less shear stress," says Kickler, an authority on hematology and blood coagulation. "What we'll need to do is help the engineers test whether their hypotheses are correct. Using the photo-activatable dyes in the system, the engineers can take hundreds of thousands of photographs and analyze how much of the activation of platelets is occurring and correlate that with shear stress. If we see less activation, that means there is less shear stress, and that would be an improvement."
Kickler and Katz, who together have more than a half-century at the university, have been energized by the new collaboration. "I've been here 27 years, and for 26 years I've had no collaboration with anyone in the medical school," says Katz. "That has changed dramatically over the last year. From our very first meeting, there were amazing dynamics; we started throwing ideas at each other. It's absolutely been an amazing project."
"I remember the first time I met with Dr. Katz," says Kickler. "He said, 'Well, we need about 100 units of blood to test this system.' I didn't know him well enough at the time to laugh. Do you know how hard it is to get 100 units of blood? … . But the collaboration has been very rewarding. I see it as not just a scientific endeavor; it meets our whole mission of education and patient care."
"To tackle a condition as recalcitrant as heart failure, we need to exploit and apply our world-class expertise across many different disciplines," says Paul B. Rothman, the Frances Watt Baker, M.D., and Lenox D. Baker Jr., M.D., Dean of the Medical Faculty, vice president for medicine, and CEO of Johns Hopkins Medicine. "We've gathered our A-team around the table. The more diverse Hopkins minds we can engage in this ambitious project, the better our prospects of bringing a revolutionary device to patients who currently have few good treatment options and, quite honestly, are overdue for a new one."
So how should an artificial heart pump blood? Should it run continuously at a steady rate, or pulsate like a real heart? Should it be made of synthetics, organic materials, or a combination of both?
Currently, most LVAD devices rely on centrifugal or axial flow pumps to circulate blood via a rotary impeller, much like a sump pump moves water out of a flooded basement. These pumps rotate at high speeds—5,000 to 10,000 rpms—in order to circulate in a minute the approximately 5 liters of blood in a human body. But, once again, all that pressure can cause problems. "It's like the force that's coming out of a water hose, and these poor little, innocent platelets that I study are very sensitive to turbulence," says Kickler.
So Katz and Sharon Gerecht, an associate professor in the Whiting School's Department of Chemical and Biomolecular Engineering, came up with the idea of using a completely different kind of pump, one that uses a peristaltic pumping mechanism—a far more gentle way of moving fluid. Peristaltic pumps rely on a symmetrical contraction and relaxation motion to generate a wave down a tube. It's basically how your gastrointestinal system transports food through the intestines. Peristaltic pumps are already used in heart/lung blood machines to circulate blood in and out of a patient during open-heart surgeries, but they have never been used in LVADs or in artificial hearts.
One of the problems with using a peristaltic pump in an artificial heart is size. The pump would need to be larger than other types of pumps because it can't move blood as quickly.
Professor of Mechanical Engineering Rajat Mittal and his graduate students have designed a series of computer simulations to look at ways they can hypothetically increase the flow rate in a smaller peristaltic pump, without causing turbulence in the system. "We've basically created this device that doesn't exist, and we can test it out with a fairly high degree of confidence," says Mittal, who heads up the Whiting School's Flow Physics and Computation Lab.
The simulations are at their earliest stages, and Mittal doesn't know if a peristaltic pump will eventually prove to be the right solution, but he says it's the right sort of thinking. "The key here is that Joe and Sharon's idea is a significant departure from conventional wisdom. I think this is exactly what we need to do. If we just follow what has been done by other teams, we end up with similar solutions. By just doing that, OK, maybe we can come up with a 10 percent better solution than what other teams have done. But I think the Hopkins team is not targeting a 10 percent improvement. We're looking for something more radical."
Another challenge for researchers is trying to map the brain-heart connection.
When you're lying down and want to get up, your brain tells the heart to beat faster, to pump more blood. Your body simply reacts. But how will a person's nervous system involuntarily control an artificial heart? "The classic example is a baseball player at the plate who isn't really doing anything," Devaney says. "But as soon as the pitcher throws the ball, a dozen different things occur automatically. Blood flow increases, there's a rush of adrenaline. It doesn't look like he's doing anything, but the body reacts to that stimulus in a way that's profoundly different than just sitting there. The mechanical heart wouldn't care that here comes a 90 mph pitch. But we want it to care. We want it to know the difference."
This is where the folks at the Applied Physics Laboratory come in. Last summer, using technology developed by APL, a Colorado man who had lost both arms 40 years ago received two modular prosthetic limbs he was able to control simply by thinking. Ultimately, a patient shouldn't have to think about controlling his heart, but the neuromuscular connection has proved doable. Also, doctors note, when they transplant a heart into a patient, the neurological connections naturally "reconnect." If an artificial heart contained enough organic material, could the body's neurological pathways reconnect with it? Or could you simply implant an artificial heart made of real muscle tissue grown from stem cells?
Sharon Gerecht has been thinking about these questions. She's an expert on stem cell differentiation and tissue engineering. The recipient of the university's first $250,000 President's Frontier Award, Gerecht has identified ways to control the fate of stem cells, coaxing them to form blood vessels—for the first time growing them in a synthetic material. She also has been able to assemble cells into small muscular networks. As far as "growing" heart muscle, the idea would be to somehow combine the two kinds of tissues—the muscular and the vascular—using pluripotent stem cells from the patient, something that has never before been done. "We can very nicely differentiate stem cells into muscle cells, but putting them together [with vascular cells] will introduce new aspects of signaling between the two cell types or tissues," she says. "It will be a challenge."
In order to begin solving some of these problems, researchers are encouraged to apply for $25,000 seed grants, funded by more than $500,000 raised so far via private donations. Devaney says he's looking forward to seeing the ideas that researchers come up with. "Some of the ideas, they can be a little crazy-sounding, but this is where we want people to go, to go out there and tinker and discover," he says. "For instance, as far as generating power, can we augment this device so it will actually capitalize on the power inside you? Maybe we can come up with a glucose-burning fuel cell. Or if we can isolate and grow heart tissue, is there any way for us to bioprint a heart muscle, vascularize it, and use it to assist a failing heart by piggybacking right off the existing heart? They sound like great ideas, but are they feasible? That's why we're trying to get people these seed grants."
Hopkins researchers are keeping a close eye on other artificial heart programs. More than a handful of research institutions are working on similar projects. In the private sector, the French company Carmat is the furthest along. Scientists there used tissue from a cow's heart to help overcome the bio-incompatibility issue with blood platelets. The patient who received one of its artificial hearts last summer went home from the hospital in January.
Still, Devaney says, the overall goals of the Hopkins Heart in terms of power, neural connectivity, and durability are far more ambitious than anything on the market today. And whatever researchers learn along the way can be used to improve current LVAD devices.
"I think the way we're approaching it systematically, it should be doable," says Kickler. "When you look up at the Sistine Chapel, you say it was a pretty big goal to paint. But the Sistine Chapel was just a series of dots. We're working on all the dots now for our masterpiece. When you look at it like that, it's not quite as daunting."
Posted in Health, Science+Technology
Tagged cardiovascular health